References
Concurrent validity of biofilm detection by wound blotting on hard-to-heal wounds
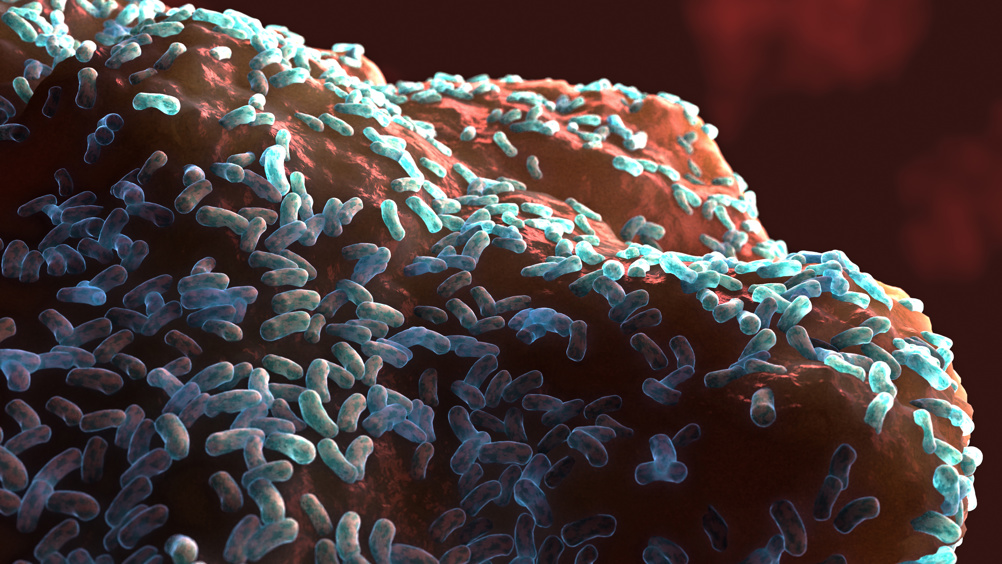
Abstract
Objective:
Wound biofilms delay healing of hard-to-heal wounds. Convenient biofilm identification tools for clinical settings are currently not available, hindering biofilm-based wound management. Wound blotting with biofilm staining is a potential tool for biofilm detection, owing to its convenience. Although predictive validity of wound blotting has been established, it is necessary to confirm its concurrent validity. Furthermore, current staining systems employing ruthenium red have some disadvantages for clinical use. This study aimed to evaluate the usability of alcian blue as a substitute for ruthenium red.
Method:
Both in vitro and in vivo clinical samples were used to investigate validity and usability.
Results:
The in vitro study showed that proteins and extracellular DNA in biofilms did not affect staining ability of ruthenium red and alcian blue in the detection of biofilms. In the in vivo study, using a wound biofilm model with Pseudomonas aeruginosa, the staining sensitivity of ruthenium red was 88.9% and 100% for alcian blue, with correlation coefficients of signal intensities with native polyacrylamide gel electrophoresis (PAGE) of r=0.67 (p=0.035) and r=0.67 (p=0.036) for ruthenium red and alcian blue, respectively. Results from clinical samples were r=0.75 (p=0.001) for ruthenium red and r=0.77 (p<0.001) for alcian blue. The sensitivities of wound blotting staining by ruthenium red and alcian blue were very high and had a good correlation with native PAGE analysis.
Conclusion:
Because the alcian blue procedure is more convenient than the ruthenium red procedure, wound blotting with alcian blue staining would be a promising tool to guide clinicians in delivering biofilm-based wound management.
Hard-to-heal wounds have become a growing healthcare problem, affecting up to 2% of the adult population (2.4–4.5 million people) in the US alone.1,2 The most prevalent types of hard-to-heal wound are vascular ulcers, diabetic foot ulcers (DFUs) and pressure ulcers (PUs), which share some features including prolonged inflammation, unresponsiveness of cutaneous cells to reparative stimuli and persistent infection due to formation of bacterial biofilms.1 A recent systematic review revealed that 78% of hard-to-heal wounds harboured biofilms.3,4 Once biofilms are well established, they cause prolonged inflammation, antibiotic tolerance and wound chronicity, eventually resulting in higher morbidity and medical costs.5,6,7 Biofilm management is an indispensable part of treatment.
Physical biofilm removal (for example, debridement, rigorous cleansing) is considered ideal to eliminate wound bioburden, giving the opportunity of a therapeutic window to expose bacteria living deep in biofilms.7,8 Unfortunately, currently available algorithms for identifying biofilm on wounds are somewhat indirect and subjective, hindering clinicians from delivering effective biofilm management.7,9,10 Moreover, during the early stage of biofilm development, referred to as critical colonisation, direct inspection is insufficient to identify biofilm presence.
Register now to continue reading
Thank you for visiting Journal of Wound Care's Silk Road Supplement and reading some of our peer-reviewed resources for healthcare professionals across Asia. To read more, please register today.